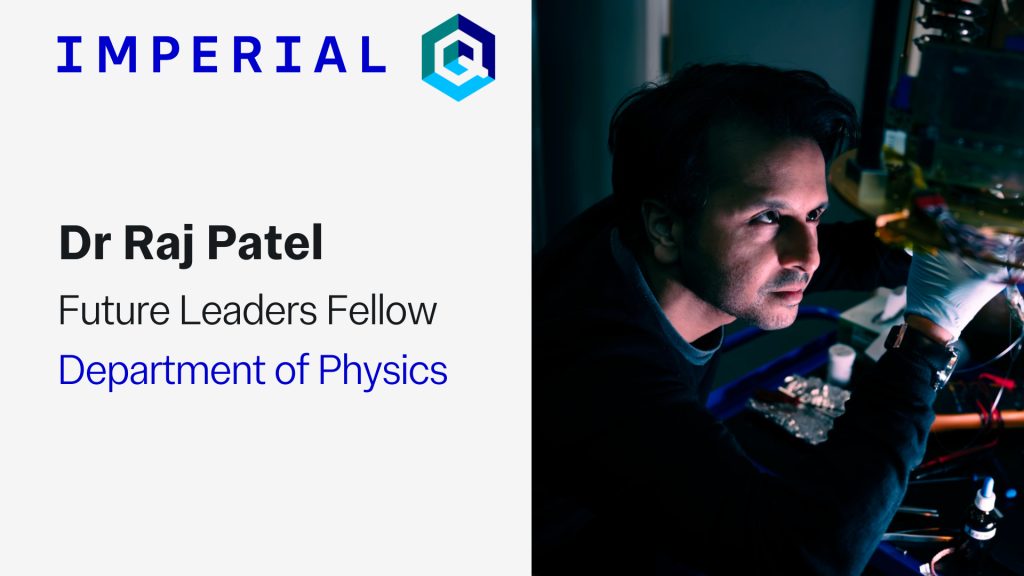
Dr Raj Patel is a Future Leaders Fellow in the Department of Physics. In this blog post, he shares more about his research as part of QuEST (Centre for Quantum Engineering, Science and Technology at Imperial College London). Dr Patel’s research group investigates experimental quantum optics and photonic quantum information technologies.
Can you tell us about your research area?
My group conducts research in experimental quantum optics and photonic quantum information technologies. Our primary focus lies in addressing the formidable challenges within the realm of photonic quantum computing, widely regarded as the most intricate and challenging of the quantum technologies. In doing so, our investigations lead us to explore not only quantum computing but also open up new possibilities in quantum communications and metrology.
In our pursuit, we harness the unique characteristics of light, a natural carrier of information that powers our global telecommunications infrastructure through fibre optic networks. Within the quantum domain, light emerges as an exceptionally versatile carrier of quantum information. It possesses the capacity for transmission over long distances and can be generated and manipulated at room temperature. Unlike their counterparts in matter, Quantum states of light remain resilient to environmental noise.
Moreover, the richness of the quantum information encoded in various properties of light—spanning spatial, temporal, phase, polarization, and frequency degrees of freedom—renders it an ideal candidate for high-dimensional quantum information encoding. Over the last four decades, quantum optics has taken the lead as a platform for experimental tests of quantum foundations and the development of emergent quantum information technologies.
The allure of quantum information lies in its ability to process and transmit information with advantages unattainable through classical means. This opens doors to computation and simulation capabilities beyond the reach of conventional digital computers, while also providing communication with superior security, with far fewer resources, than classical approaches. To fully leverage these advantages, our research requires the development of novel materials for generating quantum states of light, manipulation of these states using free-space or integrated optics, and high-efficiency detection methods, including the use of low-temperature superconducting devices.
While quantum optics forms the bedrock of our research, we recognise the intrinsic synergy between quantum optics, photonics, nanophotonics, and low-temperature measurement techniques. Armed with these experimental tools, our goal is to design and construct optical circuits capable of implementing multi-qubit logic, devise quantum algorithms addressing real-world challenges, create quantum simulators with applications in diverse fields such as chemistry, materials science, finance, and mathematics, and design circuits for error mitigation and correction in both near-term and future universal quantum computers.
What led you to study this area?
From my earliest years, I had the desire to become a physicist. This inclination persisted through the culmination of my MPhys at the University of Warwick, where my trajectory seemed destined for a future in astrophysics, specialising in supermassive black holes and galaxy formation. However, a pivotal moment transpired during a group project that investigated the state-of-the-art in quantum computing. Tasked with reviewing quantum algorithms, I found myself at the intersection of my two passions—physics and computer science.
At that time, my university did not offer courses in quantum optics or quantum information, as the field was still in its nascent stages and gaining attention from mainstream science media outlets. The groundbreaking demonstration of Shor’s algorithm by IBM, utilizing NMR, stood as a prominent showcase of experimental possibilities. Making the decision to shift my focus to quantum computing was indeed a leap of faith, given its relative novelty and the absence of established educational pathways. Yet, the potential for immediate and widespread impact of quantum computing research convinced me that this was the right direction.
Embarking on a Ph.D. journey in III-V semiconductor fabrication and quantum optics, followed by postdoctoral experiences in Australia dedicated to linear optical quantum computing, solidified my comprehension of the field. These experiences not only deepened my knowledge but also allowed me to carve out distinct research directions. Now, as I continue my pursuits at Imperial, I draw upon this diverse background to explore and advance various facets of experimental quantum optics and photonic quantum information technologies.
What are the main aims of your current research?
My current research encompasses two primary thrusts aimed at advancing the field of photonic quantum information technologies. The first stream concentrates on the development of photonic hardware with a specific focus on generating quantum states of high purity and manipulating these states with high fidelity.
In pursuit of this objective, we employ nonlinear processes in materials such as periodically-poled potassium titanyl phosphate to generate quantum light within the telecom C-band. The engineering of quasi phase-matching conditions enables the production of pure states at a wavelength conducive to low-loss transmission through both bulk and integrated optics. Recognising that photon loss poses a significant challenge in photonic quantum information processing, our efforts are directed toward minimising this source of error. Collaborating with industry partners, we are actively developing low-loss nanophotonic interconnects to mode-match these source to waveguides and optical fibres.
In parallel, we are designing programmable circuits on-chip using silicon nitride and thin-film lithium niobate. Additionally, we are utilising machine learning to improve measurements employing superconducting transition edge sensors for photon-number resolving detection.
The second stream of our research leverages the developed hardware to conduct experimental demonstrations. One goal is to employ Gaussian Boson Sampling, where squeezed states of light interfere in a large interferometer followed by photon detection, to simulate real-world molecules and address problems in statistics and graph theory. A distinct aspect of our work involves incorporating error mitigation strategies both at the hardware level and in post-processing of measurement outcomes for Gaussian Boson Sampling—a domain that has traditionally lacked such considerations. We are actively exploring ways to produce non-Gaussian quantum states of light, including Schrödinger Cat states and Gottesman-Kitaev-Preskill states. These states, with their error-correcting codes capable of addressing various errors including loss, hold the potential to serve as valuable resources for future fault-tolerant quantum computers. We aim to produce these states with the required quality and squeezing to make them viable for practical error-corrected quantum computing devices.
How could this research potentially benefit society?
The potential societal benefits of our research are multifaceted, driven by the transformative capabilities of quantum computers and the specific applications within photonic quantum information technologies.
Quantum computers hold the promise of revolutionising scientific discovery. Our recent application of Gaussian Boson Sampling to solve graph problems relevant to drug discovery has demonstrated a polynomial speed-up over classical sampling methods. As these devices scale up, we envision a future where photonic quantum computers, in tandem with high-performance computers, play a crucial role in accelerating research and development processes for new drugs. This could significantly expedite the discovery of novel pharmaceuticals, leading to breakthroughs in healthcare.
Beyond drug discovery, these devices could contribute to simulators for statistical processes relevant to finance and weather prediction. The speed and efficiency gains offered by quantum computing in these domains have the potential to revolutionise decision-making processes and enhance our understanding of complex high-dimensional systems and data.
As quantum computers emerge, posing threats to existing cryptographic protocols such as RSA, secure quantum communication becomes increasingly vital. Our research in this area aims to contribute to the development of robust and secure communication methods, ensuring the confidentiality of sensitive information in the face of evolving threats.
The hardware and techniques we are developing are not limited to quantum computing; they also find applications in quantum sensing and precision measurements beyond the shot-noise limit, with resilience to loss. These technologies could have far-reaching implications in medical imaging, spectroscopy and the precise measurement of the position, navigation, and timing of objects.
What are the next steps in your research? Are there any challenges ahead?
Moving forward, our research will focus on advancing the integration of quantum light sources, circuitry, and detectors onto a single chip. Currently, these critical components exist as separate modules, each optimised independently using materials tailored for specific functions. Connections between these modules are established through free-space or optical fibre links. While ongoing efforts to develop photonic interconnects aim to mitigate losses between components, our goal is to move towards full on-chip integration using waveguides. This not only promises to minimise losses but also ensures phase stability, enabling the exploration of applications requiring numerous quantum light sources generating resource states processed by large-depth circuits.
However, a significant challenge lies in the absence of a single material that can be monolithically processed to fulfil the specific requirements of quantum light sources, circuitry, and detection. This complexity introduces a fabrication challenge, necessitating the heterogeneous integration of materials with mismatched optical properties, leading to potential losses. Despite this obstacle, notable progress has been made in integrating nonlinear sources and single emitters with waveguide circuitry. There have also been initial attempts to incorporate superconducting detectors onto chips.
The current landscape is marked by a dynamic research environment where strides in fabrication tools and materials are continually being made. Optimism prevails that, with ongoing advancements, a fully integrated quantum photonic platform will become a reality in the future. As we address these challenges, the pursuit of seamless on-chip integration remains a pivotal step in unlocking the full potential of our quantum information technologies, paving the way for practical applications and broader adoption in various fields.
Read Research Insights with Dr Raj Patel in full