The year is 1961. Humanity is at the brink of a new era. In just a few moments, Yuri Gagarin will be the first person to break free from the Earth’s atmosphere.
This sparks the beginning of a new scientific revolution. Nearly 70 years later, space research advances our understanding of diseases like cancer, globally contributing to new medicinal technologies.
Gagarin was not just the first person to venture into space. He also made history as the first person to eat there. You might be imagining some grand, celebratory meal to mark the occasion. In reality, it all began with two words: meat paste.
Admittedly, toothpaste meat is a far cry from a Michelin-star meal. But in space, nutrition is essential for survival.
Our guest blogger, Hana Mehager, Biochemistry undergraduate student in the Department of Life Sciences, takes us on a journey to an outer space kitchen!
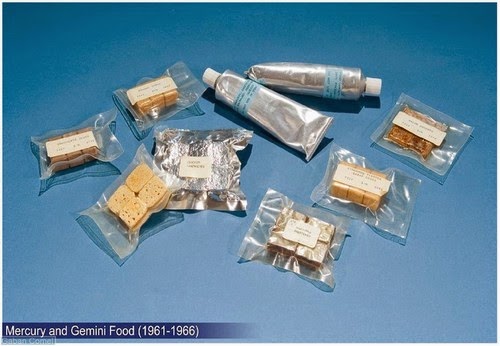
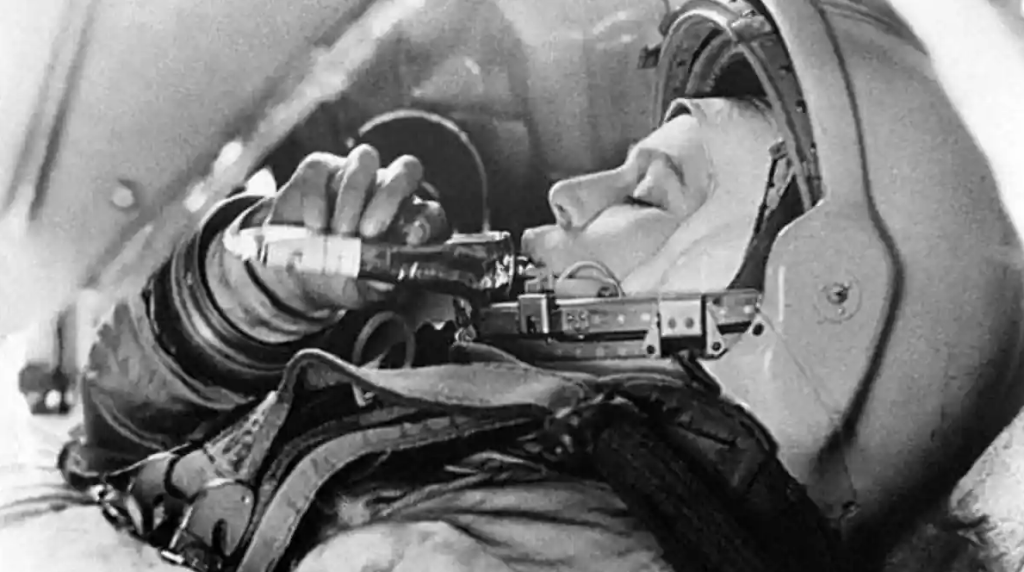