Mona Alizadeh is an undergraduate student from the Department of Physics. She spent the summer doing research at the MAST-U reactor in Culham, as part of the Undergraduate Research Opportunities Programme at Imperial. Here, she writes about the science behind her research.
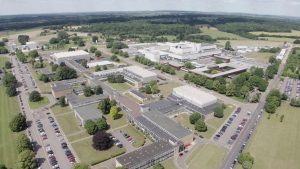
One of the biggest challenges the world is trying to solve is finding a viable replacement for fossil fuels which make up approximately 85 per cent of the world’s energy production. The current main contenders are renewables, such as wind and solar energies, as well as nuclear fission and nuclear fusion.
While nuclear fusion is still not viable as an energy source, its potential as a cleaner and almost boundless energy source makes it a strong contender to replace fossil fuels in the future. Fusion could contribute to not just a more sustainable energy future, but also a more equitable one.
My summer project was part of the Undergraduate Research Opportunities Programme (UROP) at Imperial, and was centred around magnetically confined nuclear fusion. I was extremely fortunate to be supervised by Dr Yasmin Andrew alongside Dr Omkar Myatra at the UK Atomic Energy Authority (UKAEA) and Professor Eun-jin Kim at Coventry University.
Confining the energy of the Sun
My project was focused on a specific aspect of nuclear fusion called ‘magnetic confinement’ which is essential for achieving sustained magnetically confined fusion (MCF) in tokamaks.
Fusion is the process where two light atomic nuclei come together to form a heavier nucleus. This process releases an extremely large amount of energy and is most commonly seen on stars. It is cleaner and releases more energy per reaction compared to its counterpart nuclear fission where heavy atoms split into lighter atoms.
However, to achieve fusion on Earth, we need to heat and compress a fuel (generally isotopes of hydrogen) to achieve extremely high temperatures and pressures equivalent to that found on our Sun. This is extremely difficult and thus cannot be achieved through traditional heating methods since we not only have to heat the fuel by inputting huge amounts of energy, but we also have to ensure that the plasma we create does not melt the reactor that it is contained in.
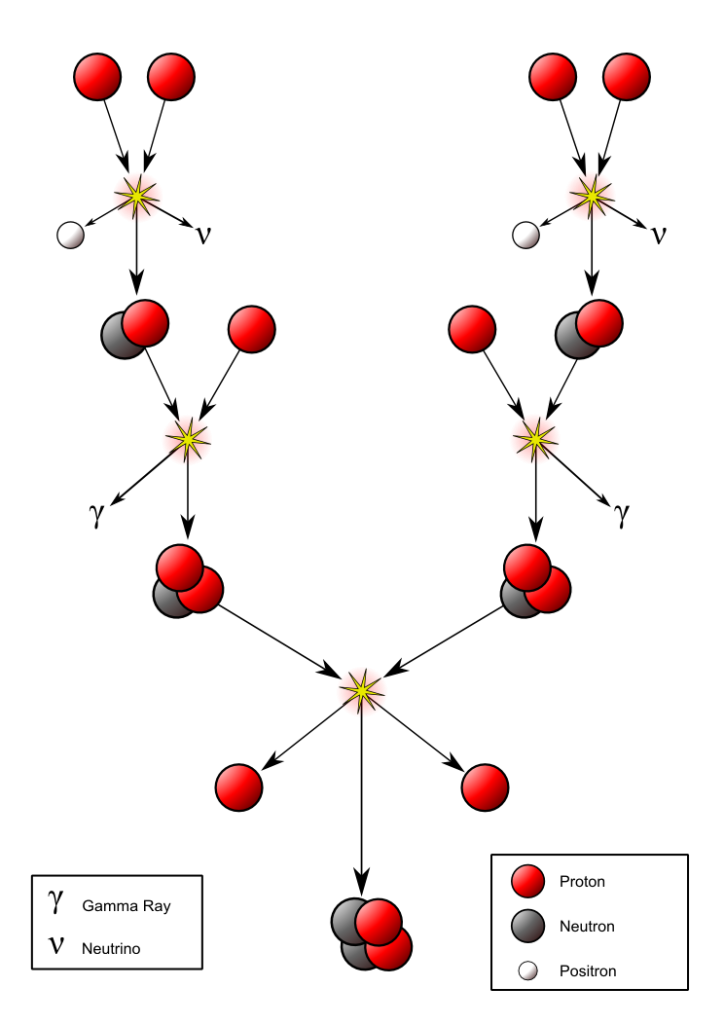
Since plasma is composed of charged ions and electrons, powerful magnets can be used to contain it. The magnetic fields keep the electrically charged particles in the plasma from touching the walls of the reactor, acting almost like a cage.
The main device used for MCF is a tokamak. There are two main types of tokamaks: the conventional tokamak shaped like a doughnut and a spherical tokamak shaped like a cored apple.
To achieve MCF, the plasma must transition from low-confinement mode (L-mode) to high-confinement mode (H-mode). In L-mode, plasma is very turbulent, like a rushing river with swirling currents that makes its flow difficult to predict or control. The super-hot plasma in L-mode does not stay neatly contained and makes it difficult to sustain a fusion reaction.
In H-mode, plasma confinement is increased within the core, so the energy stored within the confined plasma increases, transforming into heat. The transition between the two modes (L-H transition) occurs when plasma heating across a boundary exceeds a specific threshold and it can be either sharp or ‘dithering’ in nature.
My project was focused on studying the L-H transition as well as the back transition from H-mode to L-mode (H-L transition) at MAST-U, a spherical tokamak located in Culham, UK. These transitions have an immediate impact on the stability and efficiency of plasma confinement which is essential for achieving nuclear fusion.
Science behind the project
For my project, it was important to consider experiments at MAST-U that contained at least one L-H transition. To identify L-H and H-L transitions I had to visually consider changes in key plasma properties throughout each run of the reactor.
L-H transitions are identified by considering two key plasma properties. We can think of plasma as a hot soup of charged particles. Dα emission refers to the light that is emitted when deuterium ions (a type of hydrogen isotope) interact with other particles in the plasma.
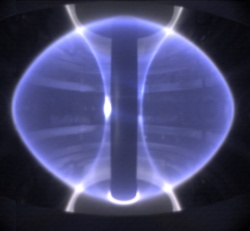
During an L-H transition there is a visible drop in this emission. It is like reducing the brightness of a lightbulb and decreased Dα emission indicates a change in plasma behaviour. Fewer particles at the edge in H-mode causes this decrease in Dα emission.
Line-integrated electron density describes how many electrons are present along a line of sight through the plasma. During a plasma L-H transition there is an increase in the line-integrated electron density as there is increased density at the tokamak core in H-mode due to greater particle confinement.
Line-integrated electron density and Dα emission are both measured through the whole experimental run so we can identify L-H transitions by looking at a graph of how these properties change through time.
I also analysed runs that had ‘Doppler back-scattering’ data. Particles in plasmas move at high speeds and understanding their motion is crucial to sustaining a fusion reaction. It is also important to understand how these particles are moving relative to one another.
You may have previously experienced the Doppler effect when an ambulance siren passes you and the sound changes pitch. This happens as the sound waves are compressed when the ambulance moves towards you and are then stretched as the ambulance moves away which is seen as a change in frequency and pitch.
The same principle applies to not just sound waves but also light waves.
We make use of the Doppler effect as a diagnostic tool to study plasma particle motion. A microwave probe beam is launched into the plasma. This beam interacts with moving plasma particles and is scattered back towards a detector. By analysing the scattered beam at the detector, we can analyse the motion of particles within the plasma. This is similar to police radars measuring car speeds by using the Doppler shifts in reflected radio waves.
Doppler back-scattering allows us to better understand particle motion in plasmas. One can analyse particle speeds, direction of particle motion and how particle motion changes with time. This information is essential for adjusting parameters of the tokamak such as magnetic field strength to maintain plasma stability and thus ensure optimal conditions for fusion.
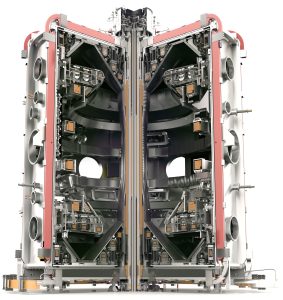
I used Doppler back-scattering data to analyse the behaviour of ‘zonal flows’ and ‘turbulence’ around the L-H transition as well as the H-L transition.
We can think of these two concepts as similar to how cars flow on a motorway. Zonal flows are organised regimes where cars smoothly travel while staying in their lanes. Meanwhile, turbulence is like a sudden traffic that causes increased collisions of plasma particles – causing the plasma to become hotter and more unpredictable.
Both concepts are important to increasing stability and efficiency of fusion reactions.
Zonal flows and turbulence are thought to have a predator-prey relationship like the delicate balances of predators and prey in wildlife.
Here, zonal flows can be thought of as predators that maintain order and control in plasmas whereas turbulence is seen as the prey that the zonal flows are trying to control. For fusion, it is essential that a balance is kept between zonal flows and turbulence.
My summer was spent studying this vital interplay between zonal flows and turbulence around the transitions to optimise H-mode as well as the L-H transition.
A summer to remember
A highlight of the summer was being able to visit the Culham Centre for Fusion Energy and see MAST-U in action. The visit was an eye-opening experience, allowing me to see cutting-edge technology and collaborative efforts in fusion research.
Hearing from the developers of data analysis and simulation software gave me a greater appreciation of the varied uses of software within research. During two experiment days at MAST-U, I had the opportunity to work alongside researchers contributing to the analysis of experimental data in real-time.
This hands-on experience was invaluable as it allowed me to apply my theoretical knowledge to real-world fusion experiments. It was inspiring to learn from experts in the field and to work as a part of the team.
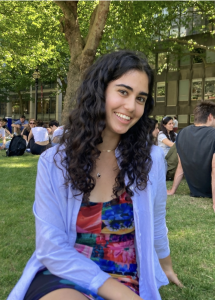
I hope to be able to further my conclusions from this project in this year’s BSc project where I can explore the open topics highlighted above.
Fusion research is fast paced with significant investment around the world. The promise it offers as an abundant and clean energy source is immense with profound implications for our current energy crisis.
However, while the dream of harnessing the power of stars is slowly becoming a tangible reality, it is important to recognise that the current challenges require significant further research. The journey to understanding plasma behaviour and mastering the L-H transition is an ongoing endeavour that demands collaborative efforts and innovative solutions.