Dr Peter Sarkies looks at how evolution can quickly come up with new mechanisms to fight infection by adapting existing processes rather than inventing new ones.
It’s January and perhaps you’ve been hitting the treadmill in the gym in an enthusiastic bid to make good that New Year’s resolution to do more exercise. To a primitive human, it’s hard to imagine a more ridiculous contraption – expending huge amounts of energy simply to stay in exactly the same place; but remarkably, this feature of a treadmill is very similar to some aspects of evolution.
The examples of evolution that are most familiar to people involve organisms adapting to their environment, with the long neck of the giraffe perfectly suited to reaching the best leaves as a famous example. Probably the most active type of evolution by natural selection occurs in response to conflicts between organisms. A good example of this is the response of species, like humans, to infection by pathogens like bacteria and viruses. Humans are engaged in a constant race to evolve new mechanisms to fight infection, because the pathogens themselves are able to rapidly adapt to become resistant to each new strategy that the host comes up with.
Evolution in the fast lane
The result of this is that the genes that are important in fighting disease generally evolve very rapidly compared to other genes – whilst the genes involved in making energy remain highly similar between humans, and even simple organisms like yeast. Many of the genes involved in immune responses have huge differences even between human and chimpanzee, and are unrecognizable if one goes back as far as fish or birds. But despite all this “running” we are still getting infected with the same pathogens, just like when after 20 minutes on a treadmill you are still in the same gym rather than 3 miles down the road!
Viruses and bacteria are examples of pathogens that get into the body from outside and are then targeted for destruction by our immune system. Amazingly, though, treadmill-like evolution also takes place within individual cells, leaving its mark on DNA. Most organisms contain within their DNA many segments called “transposable elements” or “transposons” for short. You can think of a transposon as a kind of super-lazy virus that doesn’t even bother to leave the cell. Instead, it just replicates its DNA and puts a copy somewhere else in the genome directly – that’s why it’s sometimes referred to as a “jumping gene.” This simple strategy is incredibly effective: in fact, about 40% of the human genome is made up of these elements.
As you can imagine, this onslaught places quite a burden on the cell. Marauding transposable elements could end up pasting themselves inside essential genes, which would be very dangerous. So, just as our immune system hunts and kills viruses and bacteria, cells have their own “immune systems” that are able to spot transposons and shut them down. And just like the immune system, the cell’s transposon defence mechanisms have to evolve extremely rapidly to keep up with the transposons as they evolve resistance.
Ancient defence mechanism
Our laboratory is interested in one such transposon defence mechanism known as PIWI-interacting RNA (piRNAs). piRNAs are tiny RNAs, usually between 20 and 30 nucleotides long, with similar sequences to transposable elements. piRNAs are made by the cell and are able to bind to RNAs made by transposable elements, causing them to be destroyed thus stopping the transposable elements from spreading. piRNAs are a very ancient form of genome defence, which are found in the earliest animals. Despite their ancient origin though, the way in which piRNAs are made evolves very rapidly – probably because of their role in fighting marauding transposable elements. This poses a very interesting question for understanding how piRNA production evolves to have a radically different production mechanism whilst keeping the molecule and the way in which it works the same.
Recently, we have been studying this process by investigating piRNAs in a type of animal called a nematode worm. Nematodes are best known to science through the laboratory nematode Caenorhabditis elegans. piRNAs in C. elegans have been well studied, and shown to be very unusual compared to other organisms. Usually, organisms make tiny piRNAs by chopping up a much longer RNA. Strangely, piRNAs in C. elegans are made from thousands of individual genes, which each produce one tiny piRNA. piRNA genes can be found in the genome by the tell-tale presence of a DNA sequence GTTTC very close to where the piRNA is made from. However, where this unique mechanism comes from was completely unknown because the GTTTC had not been found in other species. Additionally, how the piRNA is kept so short – when normally genes make much larger RNAs – was very mysterious.
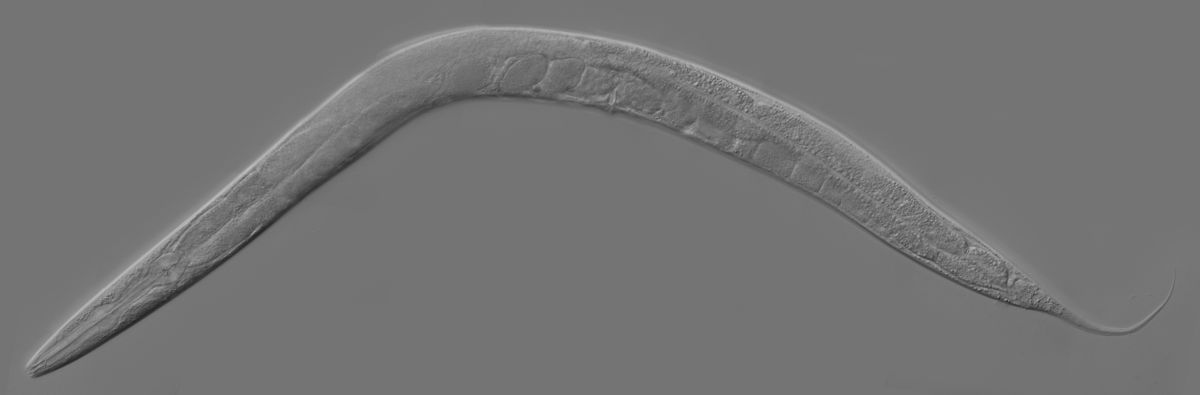
Where do nematode piRNAs come from?
We were intrigued by these problems and so decided to take an unusual approach to study them. Instead of just looking at piRNAs in C. elegans, we examined the sequences of piRNAs from a range of different nematodes spanning 400 million years of evolution. With one of these nematodes, called Plectus sambesii, the most distant from C. elegans that we studied, we struck gold. piRNA genes in this nematode had a GTTTC nearby but remarkably the GTTTC was always next to another sequence CCCGTC. We realised that the CCCGTC sequence was similar to the DNA sequence that is used in nematodes, including C. elegans, to produce a completely different type of RNA which is used in splicing genes.
The splicing RNAs are involved in a fundamental process that takes place in all organisms with a nucleus, where RNAs need to have parts chopped out of them, termed “splicing”, before they can be used to make proteins. Indeed, in Plectus, the DNA sequence near to piRNAs is exactly the same as the one used to make the splicing RNAs. Amazingly, it turned out that the proteins required to produce the splicing RNAs are also used to produce piRNAs in C. elegans! This solved the first mystery of where nematode piRNAs came from: they were “borrowed” from another type of RNA production that is much more ancient.
How are the piRNAs kept so short?
Again, studying piRNAs across nematodes came to our aid. People had previously looked for particular DNA signatures at the end of piRNA genes in C. elegans and not found anything. However, when we looked across all nematodes we found that there was a tendency for piRNA genes to be followed by a sequence rich in A and T nucleotides. These sequences have been shown before to cause the protein that makes RNA from DNA (called RNA polymerase) to come to a halt and sometimes to fall off the DNA completely. We guessed that this process might be responsible for making the piRNAs so short so we teamed up with the Meiosis lab at the LMS, led by Enrique Martinez-Perez, to test this. We took a piRNA gene in C. elegans and used a technique called gene editing to change many individual DNA bases within the A/T rich region to make them into C and G. This resulted in the piRNA being made at much lower levels, showing that the A/T rich sequence is needed to make piRNAs.
With these two findings we now have a much more complete explanation of how evolution might have come up with a completely new way to produce piRNAs in nematodes, quite quickly. First, an existing type of RNA production used to produce splicing RNAs was modified to allow it to produce piRNAs as well. Second, the piRNAs were kept short by borrowing a sequence that already had the property of being able to stop the RNA production mechanism and putting it after piRNA genes. Our study gives a good insight into how evolution can come up with apparently quite radical new mechanisms very quickly by adapting existing processes rather than inventing new ones from scratch. We think that this kind of process is very likely to explain how such diverse mechanisms of transposon defence evolved across animals.
Dr Peter Sarkies is a Senior Lecturer at Imperial College London and Head of Epigenetic Inheritance & Evolution at the MRC London Institute of Medical Sciences. The work in his laboratory is funded by the MRC.
The group’s recent paper ‘Comparative epigenomics reveals that RNA polymerase II pausing and chromatin domain organisation control nematode piRNA biogenesis’ was published in Developmental Cell on 31 January 2019.